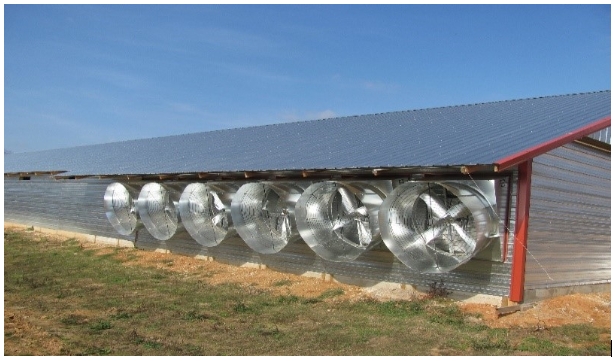
While providing on average 17 percent of food calories and more than a third of protein to human diets (Herrero et al., 2009), livestock consumes almost 60 percent of the total global biomass harvest (Krausmann et al., 2008), uses around 30 percent of agricultural water withdrawals (Mekonnen and Hoekstra, 2010; Peden et al., 2007), and dominates the agricultural nitrogen cycle (Bodirsky et al., 2012, 2014; Bouwman et al., 2013).
However, livestock farming reduces vulnerability to hunger from environmental risks for 600 million poor smallholder farmers and their families (Steinfeld et al., 2006; Thornton and Herrero, 2010) and provides other services beyond food production (Herrero et al., 2009; Steinfeld et al., 2006).
Food Security
Food security is critical to achieving sustainable growth, poverty reduction, and political and economic stability, especially in regions of the world undergoing rapid population growth, such as sub-Saharan Africa, where population is projected to double by 2050 (UN, 2020). In 2018, total population of sub-Saharan Africa was approximately 1.08 billion; and may grow to 2.7 billion by 2060 (UN, 2020). Smallholder farmers provide up to 80 percent of the food supply in sub-Saharan Africa through cropping and livestock production (Tabler et al., 2020) and play a major role in food security in developing countries (Dixon et al., 2004).
Although it impacts on many critical environmental impacts, including greenhouse gas emissions, livestock production is crucial to the human protein supply, particularly for many poor and undernourished people in the developing world (Weindl et al., 2015). As a result, livestock is intertwined with many aspects of the challenge to sustainably feed a growing population and achieve a balance between livelihoods, food security and the environment (Herrero and Thornton, 2013). Therefore, the need exists to fill large gaps in knowledge of how climate change could affect livestock production and how a transformation of livestock production systems could contribute to climate-smart agriculture and energy-smart farming.
Heat Stress
In OECD countries, poultry and swine production occurs predominantly inside livestock confinement buildings (Gerber et al., 2013), characterized by a mechanical ventilation system, high stocking density, and insulation (Schauberger et al., 2019). The increase in heat stress for farm animals due to anthropogenic global warming in recent decades is well known, especially in livestock confinement buildings. These effects are relevant not only from an animal welfare perspective (Huynh et al., 2005) but also from an economic point of view (St-Pierre et al., 2003).
Heat stress directly impairs on productivity of meat, milk, egg yields and quality as well as reproductive performance, animal health, and welfare. This sensitivity is caused primarily by the higher indoor temperatures compared with outdoor temperatures due to the highly sensitive heat load of the animals. Therefore, future global warming will affect requirements for the control of thermal conditions of livestock buildings, such as through mechanical ventilation systems (Olesen and Bindi, 2002). Such adaptation measures can help reduce heat stress and its impact on welfare, health, and productivity. While these measures will increase the direct input costs associated with intensive livestock production, they should be partly offset by the beneficial effects on livestock productivity and animal welfare.
Adaptive Measures and Technologies
Adaptive measures that include floor cooling, higher air velocity at animal level to increase convective heat releasee (known as the wind chill effect), tunnel ventilation, booster fans, hybrid ventilation systems, and cooled drinking water may influence the immediate thermal vicinity of the animals. Of all these adaptive measures, tunnel ventilation is likely the most widely used, particularly in the poultry and swine industries. Fans are essential components of mechanically ventilated poultry houses, swine barns, and dairy sheds. Fan performance characteristics, especially power consumption, are critical for the optimization of environment control and energy conservation. Attention should be focused on improving the energy efficiency of tunnel ventilation fans, with shutter type and location key to an energy efficient system and to optimize overall fan performance. Traditional shutters may be located in the interior (intake side; Fig 1) or exterior (exhaust side) of the building. Exterior butterfly shutters (Fig. 2) are significantly better than traditional interior or exterior shutters.
They offer little resistance to the flow of air in to or out of a fan. In addition, the fan does not have to work against gravity to open the shutter and performance can be further improved because dust and dirt buildup doesn’t increase the force required to open a butterfly shutter as it does with traditional shutters. As a result, fans with butterfly shutters are 5 to 10 percent more efficient than clean traditional shutters and 10 to 20 percent more efficient than dirty traditional shutters. However, butterfly shutters are not without their issues.
They tend to scare animals when they “slam” close when fans shut off and they allow more light into the house, which may be detrimental when lights are dimmed. However, the most significant issue is that they are prone to condensation problems that can cause moisture to pool at the bottom of the fan and run down interior walls, possibly damaging the fan and the wall over time. Therefore, because of the condensation issues, butterfly shutters are better suited for warmer climates.
Fan performance has been greatly improved by installing discharge cones on tunnel fans (Fig. 3) that. reduce the exit pressure on fans and tend to increase the air flow approximately 5 percent without increasing power demand. Yet another way to improve fan performance is to increase the size. All other things being equal, larger fans are more efficient than smaller fans, although it is useful to understand some basic fan laws. For example, the volume of air flow (cubic feet per minute; cfm) is proportional to fan speed; increasing fan speed by 10 percent increases fan output by 10 percent.
Installing a larger motor pulley to speed up the fan blades would increase the fan air volume movement capacity, but since power demand is exponentially related to fan speed, increasing it by 10 percent will increase power demand by around 30 percent. Therefore, installing a bigger pulley to move more air will eventually burn out the electric motor driving the fan unless a higher capacity motor is installed.
However, fan laws work both ways. If fan speed is decreased by 10 percent, power usage is decreased by 30 percent. It may be more energy efficient to install more fans with a smaller pulley moving less air than installing fewer fans with a larger pulley moving more air. An alternative to changing pulleys would be to install variable speed motors and controllers. Variable speed, direct drive fans offer several advantages including power savings of 30 to 50 percent. They also clean easily, come with a motorized butterfly shutter, are well insulated, and are much quieter when running at full speed.
However, there are disadvantages to direct drive, variable speed fans as well. Initial cost is at least twice that of a comparable belt driven fan. In addition, some controllers are not capable of controlling multiple variable speed motors. There are more electronic components which may mean more risk of lightening damage. Variable speed fans may also have a problem operating at higher static pressures when running at low speeds. Most variable speed fans move 50 percent of their rated airflow volume at approximately 65 percent of their full speed rotations per minute (Harmon et al., 2012).
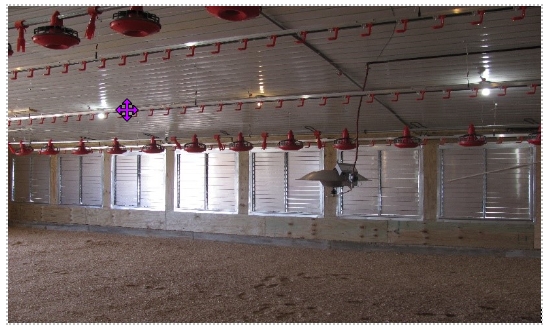
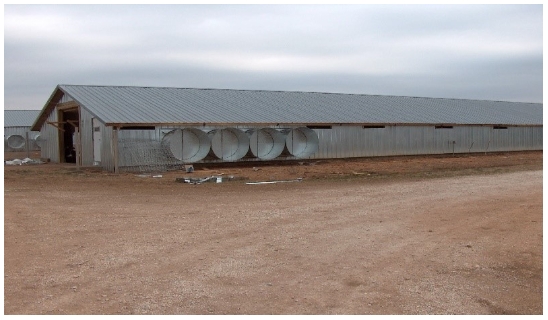
Fan Selection and Maintenance
Fans should be selected based on air flow and efficiency ratings. Choose fans that are in the upper 25 percent of rated fan efficiencies. The Bioenvironmental and Structural Systems (BESS) Laboratory at the University of Illinois tests all major agricultural fans with results presented on their website (http://bess.illinois.edu/index2.htm). All manufactured fans should be tested by such an independent laboratory to indicate air flow and efficiency as a function of static pressure. A fan should not be selected on its diameter alone and it cannot be assumed that two fans of equal size will have equal performance. Different motors, blade curvature, and other attributes greatly affect performance.
Fans must be clean to be most efficient. Regular cleaning and maintenance of fans and fan accessories is essential to maintaining fan performance. Guard screens, shutters, and other accessories as well as fan wear and tear affect airflow rate and efficiency. There are significant differences in power consumption between fans before and after cleaning as well as considerable variation in both airflow and power consumption among otherwise identical fans. In addition, maintenance of proper tension on drive belts and replacement of worn belts in a timely manner were found to be important in maintaining fan performance. A small reduction in fan speed from a worn or slipping belt has a large effect on airflow, which increases power consumption and costs. Therefore, while having the right equipment is important, it requires good maintenance to make energy-smart farming efficient and sustainable.
References
Bodirsky, B. L., A. Popp, I. Weindl, et al. 2012. N2O emissions from the global agricultural nitrogen cycle—current state and future scenarios. Biogeosciences, 9:4169–4197.
Bodirsky, B. L., A. Popp, H. Lotze-Campen, et al. 2014. Reactive nitrogen requirements to feed the world in 2050 and potential to mitigate nitrogen pollution. Nat. Commun. 5:4858. Available at: https://www.nature.com/articles/ncomms4858.pdf. (Accessed: 10 June 2021).
Bouwman, L., K. K. Goldewijk, K. W. Van Der Hoek, et al. 2013. Exploring global changes in nitrogen and phosphorus cycles in agriculture induced by livestock production over the 1900-2050 period. Proc Natl. Acad. Sci. USA. 110(52):20882-20887.
Dixon, J., A. Tanyeri-Abur, and H. Wattenbach. 2004. Framework for analysing impacts of globalization on smallholders, Smallholders, globalization, and policy analysis. Available at: http://www.fao.org/3/y5784e/y5784e02.htm#bm02. (Accessed: 8 June 2021).
Gerber, P., H. Steinfeld, B. Henerson, et al. 2013. Tackling climate change through livestock. A global assessment of emissions and mitigation opportunities. Food and Agriculture Organization of the United Nations (FAO), Rome.
Harmon, J., M. Brumm, and D. Petersen. 2012. Managing swine ventilation controller settings to save energy. Iowa State University Extension and Outreach PM 2089T. March.
Herrero, M., P. K. Thornton, P. Gerber, and R. S. Reid. 2009. Livestock, livelihoods and the environment: understanding the tradeoffs. Curr. Opin. Environ. Sustain. 1:111-120.
Herrero, M., and P. K. Thornton. 2013. Livestock and global change: emerging issues for sustainable food systems. Proc. Natl. Acad. Sci. USA. 110:20878-20881.
Huynh, T. T. T., A. J. A. Aarnink, W. J. J.Gerrits, et al. 2005. Thermal behaviour of growing pigs in response to high temperature and humidity. Appl Anim Behav Sci. 91:1–16.
Krausmann, F., K. Erb, S. Gingrich, C. Lauk, and H. Haberl. 2008. Global patterns of socioeconomic biomass flows in the year 2000: A comprehensive assessment of supply, consumption, and constraints. Ecol. Econ. 65:471-487.
Mekonnen, M. M., and A. Y. Hoekstra. 2010. The Green, Blue, and Grey Water Footprint of Farm Animals and Farm Products. UNESCO-IHE. Delft, the Netherlands.
Olesen, J. E., and M. Bindi. 2002. Consequences of climate change for European agricultural productivity, land use and policy. Eur J Agron. 16:239–262.
Peden, D., G. Tadesse, A. K. Misra, et al. 2007. Water and livestock for human development, In D. Molden (Ed), Water for Food, Water for Life: A Comprehensive Assessment of Water Management in Agriculture, Oxford: Oxford University Press, pp 485-514. Available at: http://www.iwmi.cgiar.org/assessment/Water%20for%20Food%20Water%20for%20Life/Chapters/Chapter%2013%20Livestock.pdf. (Accessed: 8 June 2021).
Schauberger, G., C. Mikovits, W. Zollitsch, et al. 2019. Global warming impact on confined livestock in buildings: efficacy of adaptation measures to reduce heat stress for growing-fattening pigs. Clim. Chang., 156:567-587.
Steinfeld, H., P. Gerber, T. Wassenaar, et al. 2006. Livestock’s Long Shadow: Environmental Issues and Options. Food and Agriculture Organization of the United Nations (FAO). Rome.
St-Pierre, N. R., B. Cobanov, and G. Schnitkey. 2003. Economic losses from heat stress by US livestock industries. J. Dairy Sci. 86:E52-E77.
Tabler, T., M. L Khaitsa, S. H. Mbaga, J. N. Jeckoniah, J. Moon, and J. Wells. 2020. Gender issues in ag Extension programming in sub-Saharan Africa. Mississippi State University Extension Service, Publ. No. 3501. August.
Thornton, P. K., and M. Herrero. 2010. Potential for reduced methane and carbon dioxide emissions from livestock and pasture management in the tropics. Proc. Natl. Acad. Sci. USA 107:19667-19672.
U.N. (United Nations). 2020. United Nations: Population. Available at: https://www.un.org/en/sections/issues-depth/population/index.html#:~:text=Africa%3A%20fastest%20growing%20continent,projected%20to%20double%20by%202050. (Accessed: 8 June 2021).
Weindl, I., H. Lotze-Campen, A. Popp, et al. 2015. Livestock in a changing climate: production system transitions as an adaptation strategy for agriculture. Environ. Res. Lett. 10:094021.